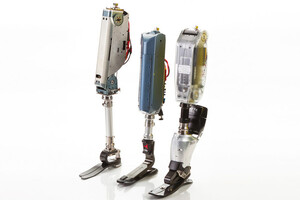
In February of 2014, a medical team at the University of Michigan’s C. S. Mott Children’s Hospital, in Ann Arbor, carried out an unusual operation on a three-month-old boy. The baby had been born with a rare condition called tracheobronchomalacia: the tissue of one portion of his airway was so weak that it persistently collapsed. This made breathing very difficult, and it regularly blocked vital blood vessels nearby, including the aorta, triggering cardiac and pulmonary arrest. The infant was placed on a ventilator, while the medical team set about figuring out what to do. The area of weak tissue would somehow need to be repaired or replaced—a major and dangerous operation in so small a patient. The team consulted with the baby’s doctors at Akron Children’s Hospital, in Ohio, and they soon agreed that they had just the right tool for this delicate, lifesaving task: a 3-D printer.
A 3-D printer used by researchers at Harvard University’s Wyss Institute creates a model vascular network.CREDITCOURTESY LORI K. SANDERS
As its name suggests, a 3-D printer prints ink not on a flat substrate, such as paper, but in three dimensions, in successive layers; the ink is substrate and substance in one. The first 3-D printers were developed in the nineteen-eighties, by an American engineer named Charles Hull. The “ink” was an acrylic liquid that turned solid when exposed to ultraviolet light, typically from a laser beam. Makers of cars and airplanes could design complicated parts on a computer and then print out prototypes for manufacture; now they often print the part, too. Three-dimensional printers have become inexpensive and ubiquitous. Staples and Amazon now offer 3-D printing services, and the list of 3-D-printed products generally available includes nuts, bolts, earbuds, eyeglasses, athletic cleats, jewelry, cremation urns, “Star Wars” figurines, architectural models, and even entire houses. In the United States, debates have erupted over whether citizens should be allowed to 3-D-print handguns at home, which the technology makes possible. Today’s printers print in plastics, and also in silver, gold, and other metals, along with ceramics, wax, and even food. (NASA is working on a zero-gravity 3-D printer that can make pizza for orbiting astronauts.) For a small fee, you can upload a photograph of your face and receive back your likeness in the form of a 3-D-printed bobblehead doll.
The medical procedure at the University of Michigan worked on a similar principle. The researchers began by taking a CT scan of the baby’s chest, which they converted into a highly detailed, three-dimensional virtual map of his altered airways. From this model, they designed and printed a splint—a small tube, made of the same biocompatible material that goes into sutures—that would fit snugly over the weakened section of airway and hold it open. It was strong but flexible, and would expand as the boy grew—the researchers likened it to “the hose of a vacuum cleaner.” The splint would last for three years or so, long enough for the boy’s cells to grow over it, and then would dissolve harmlessly. Three weeks after the splint was implanted, the baby was disconnected from the ventilator and sent home. In May of 2013, in The New England Journal of Medicine, the researchers reported that the boy was thriving and that “no unforeseen problems related to the splint have arisen.”
This sort of procedure is becoming more and more common among doctors and medical researchers. Almost every day, I receive an e-mail from my hospital’s press office describing how yet another colleague is using a 3-D printer to create an intricately realistic surgical model—of a particular patient’s mitral valve, or finger, or optic nerve—to practice on before the actual operation. Surgeons are implanting 3-D-printed stents, prosthetics, and replacement segments of human skull. The exponents of 3-D printing contend that the technology is making manufacturing more democratic; the things we are choosing to print are becoming ever more personal and intimate. This appears to be even more true in medicine: increasingly, what we are printing is ourselves.
This past June, I attended the Aspen Ideas Festival, in Colorado, which opened with a focus on innovations in health. The first speaker was Scott Summit, a tall, bearded industrial designer for a company called 3D Systems. The company was started by Charles Hull, and has since grown into one of the world’s leading purveyors of 3-D printers and services. The company contributed to the design of a popular product called Invisalign, an alternative to the metal braces used in orthodontics. Treatment begins with a scan of the patient’s bite, to determine how it might be fixed over time. Then an individualized “aligner,” which looks like a clear plastic mouth guard, is printed for the patient to wear. Periodically, the design is adjusted and a new aligner is printed, until the problem is corrected.
3D Systems has made steady inroads into the medical market. A few months ago, the company, together with researchers at Children’s Hospital Oakland, completed an early test of a new kind of spinal brace for young adults with scoliosis. To correct the disorder, the typical brace must be worn during virtually every waking hour, but most kids can’t stand to do so. “If you look at the braces now, they press against the body,” Summit told me over the phone. “They come with Velcro straps; they’re hot in the summer. Most teen-agers don’t want to walk around looking like that.” I could sympathize. Several years ago, after a spinal-fusion operation, I had to wear a similar brace for months, and the experience was torture—the brace was highly uncomfortable and impossible to disguise. Summit’s new brace looked, instead, like a formfitting lace tank top. It was made from finely ground nylon powder that had been precisely melted, then left to solidify into a filigree pattern. The end result was light, breathable, and customized to the wearer’s body and medical needs, and could be easily worn under clothing. The company tested the brace with twenty-two girls, and is working toward making it more widely available.
At Aspen, Summit appeared onstage with a forty-six-year-old wheelchair-bound woman named Amanda Boxtel. In 1992, a skiing accident left Boxtel unable to use her legs; she is now the director of Bridging Bionics, a foundation that tries to restore mobility to people who are paralyzed. In 2013, researchers at 3D Systems scanned the contours of Boxtel’s lower body and then printed snug sleeves, made of flexible nylon fibres, for her torso, thighs, and shins. They then connected these to an existing set of motorized leg braces and hand controls made by a company called Ekso Bionics. The result, in effect, is a customized exoskeleton; when Boxtel wears it—as she later did at the conference—she can slowly walk. Other motorized mobility aids exist, Summit said, but because they aren’t personally sculpted, the wearer risks getting abrasions and infections when the device puts pressure on the hips and legs. “I love my robot,” Boxtel told me later. “It was made from me and for me. But I want more. I want to think of it as my sleek and sexy sports car.”
Until fairly recently, most 3-D-printed medical devices were aimed at shoring up the human body from the outside, but, increasingly, they are being slipped into us as well. 3D Systems supplies its printing technology to a company called Conformis, which prints more than a thousand customized knee implants a year. (Although the market for customized knee implants is surging, the jury is still out on whether they provide a better outcome than generic implants do.) Earlier this year, surgeons in Wales used a 3-D printer to reconstruct the facial bones of a twenty-nine-year-old man named Stephen Power, who fractured his left cheekbone, eye sockets, upper jaw, and skull in a motorcycle accident. The medical team scanned Power’s skull and, based on the unbroken bones, determined what his full facial structure should be. They then printed a replica in titanium and successfully implanted it.
Recently, I spoke with Dr. Oren Tepper, the director of craniofacial surgery at Montefiore Medical Center, in the Bronx, who has found an innovative use for 3-D printing in his practice. In 2012, he was presented with an infant girl named Jayla, who had been born with only a rudimentary jaw. The condition made it difficult for her to breathe; the next step would have been to give her a tracheostomy. The usual solution, a full jaw reconstruction, would have required numerous, risky bone-graft surgeries and can’t be performed until a child is older.
“I just hope people in the future are, like, ‘What the hell are these things?’
Instead, Tepper made a full CT scan of Jayla’s head, and from that information arranged to have 3-D-printed a detailed plastic model of her ideal jaw. The model wouldn’t replace her existing jaw; rather, Tepper would transform her existing jaw into one very similar in shape to the model. Tepper then had printed something akin to a three-dimensional stencil that fit exactly around the lower part of her face; it had slits and holes in it to indicate where he could drill without damaging her facial nerves. Finally, he attached a ratchet to her jaw; each day, he tugged her jaw forward by a millimetre, allowing her bone cells to grow and fill in the stretched region. When the whole process was complete, many weeks later, Jayla had a normal-sized jaw. Tepper now treats two or three children with similar malformations each year.
“I’m from the younger generation, comfortable with new technology,” he told me. “You could try to do such a complex surgery without virtual modelling, and without 3-D printing. But it would be much more challenging, much more risky, with much more opportunity to fail.”
The biggest leap for medical 3-D printing lies ahead. For years, researchers have dreamed of engineering kidneys, livers, and other organs and tissues in the lab, so that a patient who needs a transplant doesn’t have to search for a donor. But growing usable tissue in the lab is notoriously difficult; the advent of 3-D printers that can print ink made of cells has offered a ray of hope. In the early nineteen-nineties, Anthony Atala, the director of the Wake Forest Institute for Regenerative Medicine, began growing human bladder cells on biodegradable scaffolds in his lab. The cells formed a kind of pouch, which he successfully implanted around the bladders of seven children with poor bladder function, relieving their condition. That achievement was soon followed by a cascade of announcements declaring victory in the race to create a true human organ. Most of these projects involved growing tracheal cells or cardiac cells or kidney cells on polymer scaffolds, often produced with 3-D printers, but none have succeeded in growing into full-fledged organs. As scientists make more concerted efforts to grow organs in the lab, the question is no longer whether they will succeed but how.
The first microscopes were invented in the sixteenth century, around the time of the invention of the telescope. Two realms were soon revealed: the macrocosmic world of celestial bodies and the vast distances between them, and the intimate, interior world of microscopic organisms and cells. But, whereas astronomers readily grasped that the mechanics of the universe proceed in three dimensions, cell biologists often still seem stuck in a two dimensions. Partly that’s a function of how most microscopes work: a specimen must be placed on a thin glass slide so that it can be illuminated from above or below. As a result, even with modern computers and mapping software, biologists struggle to understand how our cells interact with one another to form three-dimensional tissues and organs, and they’ve had an even harder time re-creating those geometries.
In my lab, for example, I study endothelial cells, which line the insides of our veins, arteries, and capillaries. When these cells are removed from the body, they quickly die; growing and sustaining them in a lab requires special procedures and equipment. First, the cells are placed on a plastic dish coated with a gelatinous mix of collagen and other proteins. Then the dish is placed in an incubator, which is set at a certain temperature and infused with just the right amounts of oxygen, nitrogen, carbon dioxide, and water vapor, in an effort to approximate the ambient conditions within a living body. The cells survive for a few weeks, but I can’t control how they organize themselves in the collagen matrix. I have yet to place a dish of endothelial cells in the incubator and return several days later to find a working blood vessel.
It’s hard to overstate the importance of structure to the proper functioning of a biological system. Sickle-cell anemia is caused by a single, shape-altering gene mutation. The normal gene codes for a protein called globin, which helps red blood cells carry oxygen to the body’s tissues. When the gene mutates, however, the resulting protein collapses on itself and can clog the blood vessels. Until recently, Alzheimer’s researchers did not have reliable ways of studying how brain cells spontaneously make the abnormal amyloid proteins that are thought to be at play in the disease. Neurons raised in petri dishes simply don’t behave the way they do in the brain. But in October, in an article in Nature, a team of scientists at Massachusetts General Hospital reported that when they grew the neurons in a gel matrix, so that the cells could interact with one another in three dimensions, they saw a far more realistic representation of the disease—a potential boon for future lab research on Alzheimer’s.
Efforts to engineer tissues and organs have been similarly hampered by two-dimensional constraints. Jordan Miller, a bioengineer at Rice University, has noted that a viable replacement organ will need to be made of at least a billion working cells, of many different types. “Scaling up tissue constructs is first and foremost a numbers game,” Miller wrote recently in the journal PLOS Biology, in a paper titled “The Billion Cell Construct: Will Three-Dimensional Printing Get Us There?” A flat petri dish, or several of them, won’t suffice; the cells need to be organized in such a way that they can exchange nutrients, growth factors, and other information.
But the expertise for wrangling such numbers, Miller wrote, “is still off by several orders of magnitude.” Scientists have typically tried to address the challenge by growing different cell types atop plastic or epoxy scaffolds. These masses soon become tombs; as the cells on the outside proliferate, those on the inside, starved of nutrients and deprived of oxygen, die. One could conceivably grow billions of kidney cells, and even make them look like a kidney, but, without a growing vasculature to nourish the whole mass, the behavior of that mass will bear little resemblance to that of an actual kidney.
“This is an area that has a lot of hype,” Jennifer Lewis, a materials scientist at Harvard, told me recently. “I remember the first time I saw a TED talk: I started watching the video, and the guy announced, ‘We’re printing a kidney!’ Then he showed some material in the shape of a kidney. I thought it was misleading, printing the shape but presenting it as if it were a kidney. We don’t want to give people false expectations, and it gives the field a bad name.”
Lewis is fifty, with short brown hair, rimless glasses, and a friendly, focussed manner. Although she likes to dampen any expectations about the 3-D printing of organs and tissues, her work is becoming central to the emerging field. In February, Lewis and a graduate student, David Kolesky, and other members of their research group published a paper in Advanced Materials describing a potential way to keep large masses of cells thriving. With a customized 3-D printer, they were able to print a protein matrix and living cell types in a pattern similar to what is found in the body. Critically, they managed to create within these blocks of tissues a network of vascular channels that, much like blood vessels, can deliver nutrients to the cells and keep them alive. It isn’t 3-D-printed organs, but it is a vital advance toward that goal. “We call it 3-D bioprinting,” Lewis told me, with an emphasis on “bio.”
Lewis grew up in Palatine, Illinois, and went to college at the University of Illinois at Urbana-Champaign. As a freshman, she was recruited into the ceramic-engineering program; she stayed with it and eventually, at M.I.T., got her doctorate in ceramics science. Lewis said that she likes ceramics for their unusual properties. They can form glass, porcelain, and clay, but they also readily conduct electricity and are a key material in many high-tech electronics.
“Ceramics processing has always been part science, part art,” she told me. “What I found most fascinating was that a given material’s properties can vary widely depending on how it is assembled.” She added, “I fell in love with the idea of creating matter that matters.”
In 1990, Lewis returned to Urbana-Champaign to teach, and began to work on 3-D printing, which she considered the perfect tool for constructing materials “voxel by voxel”—volume element by volume element. “If you think about 3-D printing thirty years ago, it was focussed on ultraviolet-curable resins, or thermoplastics, and it was largely a prototyping tool to make shapes or forms,” she said. “I wanted to create functional materials and devices, rather than simply making prototypes.”
In 2001, she began collaborating with Scott White, an accomplished materials engineer who for years had been working to create plastics and other building materials that would mend themselves when damaged. White told me that the kinds of cracks that can compromise the plastic or metal components in a car or an airplane typically occur within the material and aren’t easily detected from the outside. White and his colleague Nancy Sottos had found a multi-step solution to the problem. First, with a 3-D printer, they created materials populated with microcapsules that, in turn, were filled with special healing agents; as the material suffered wear and tear, the microcapsules would break open and release their contents. The contents were monomers, simple plastic molecules that, when they encountered a certain other chemical that was embedded in the material, would react to fill a potential crack.
“You’re getting a legendary desk. Some of these cords go back six, seven managers ago.
Second, once Lewis joined the team, the researchers realized that the material should have microchannels in it, so that the healing agents could more easily reach the cracks, just as clotting proteins and platelets in the body travel through capillaries to reach and heal open wounds. At first, they used a wax-based ink that would melt when heated. In 2011, Lewis went about developing Pluronic ink—a material that is gelatinous at room temperature but, counterintuitively, turns to a liquid when it is cooled to just above freezing. With their 3-D printer, Lewis and White could then make plastic objects that were embedded with intricate networks of the Pluronic-ink gel. Afterward, the object could be cooled down and the liquified ink could be sucked out, leaving a nexus of channels. For this reason, Lewis often refers to the material as “fugitive ink.”
“It’s a bio-inspired approach to creating materials that can heal themselves,” Lewis said. “My big role in the project was to find ways to use 3-D printing to embed this microvascular network. Once we did that, it was pretty easy for me to see the broader implications.”
In 2013, Lewis left Urbana-Champaign and took up a faculty post at Harvard, doing research at the Wyss Institute for Biologically Inspired Engineering. Her laboratory has several dozen undergraduates and graduate students working in it and occupies the better part of a new concrete-and-glass building on a quiet street just north of the main campus. Central to the lab’s work are three customized 3-D printers, each worth a quarter of a million dollars. When I visited Lewis in September, I told her I was eager to see one.
Lewis led me through a warren of corridors and offices to a room where one of the printers sat on supports. It was immense. The base of the printer was a granite block five feet long, four feet deep, and a foot high, weighing a ton and a half. The printer does such fine-scale work that a stable base is essential, Lewis said. Resting on the block was a flat stage or platform, above which, in a vertical row, stood four rectangular steel containers, each a foot or so tall—the ink dispensers. They resembled the tubes of nuts in the bulk-foods section of the grocery store, although each of these dispensers ended at the bottom in a small, conical plastic tip, like that of a pipette. A tangle of colored wires connected the dispensers to some machinery behind them, and each dispenser was controlled at the top by a robotic arm. To the side sat a large monitor and a computer, which controlled the printer.
Each dispenser contained a different biological material, Lewis explained. One held an aqueous suspension of chemically treated collagen, which serves as the matrix on which many of the body’s tissues take shape. Two others held suspensions of fibroblasts, the gristly cells that form the body’s connective tissue. (The fibroblasts were harvested from discarded neonatal foreskins, which many hospitals save for use in research.) The last dispenser contained the fugitive ink that Lewis had developed to create channels within materials. David Kolesky, the graduate student who was working with Lewis, then demonstrated how it all worked.
First, he placed a clean glass slide on the platform, under one of the dispensers. On the computer, he called up a software program and found an image representing the block of tissue that he would be printing. It looked like a rectangle of semi-clear gelatin, within which was a vascular network: a channel entered at one end and branched into smaller vessels, which looped around and ultimately joined back into a single vessel that exited at the other end. It was a simple network, approximating the way that an artery divides into smaller capillaries that eventually recombine into a vein. “You can design whatever vascular pattern you want,” Kolesky said. He showed me another slide, with a more complex branching pattern; it looked like a leafless tree branch. I recognized it: it was modelled on the pattern of blood vessels that supply oxygen and nutrients to the surface of the heart.
Then Kolesky hit “Run.” The dispenser with the fugitive ink moved quickly and almost imperceptibly, releasing an exceptionally thin stream of what looked like agar onto the glass slide. The printer clacked and clattered like a busy riveting machine. In a minute or so, the job was done; the printer had left a trail of gelatinous ink that exactly matched the pattern on the computer. The stream of ink was about a tenth of a millimetre in diameter, and the entire pattern covered an area a little larger than a matchbook.
The printer wasn’t rigged to finish the job, but Kolesky explained what would typically happen next. The other ink dispensers would take their turn, laying down a lattice of collagen and fibroblasts that would solidify around the network of fugitive ink, encasing it in tan-colored living tissue. To drain the fugitive ink, Kolesky would place the tissue on a chilled stone cube; this would cause the ink to change from a gel to a liquid, after which he could then extract it with a small suction device. The end result would be a block of living tissue suffused with intricate vessels capable of carrying nutrients to the cells within.
The last step was to me the most remarkable. Once the vessels were empty, Kolesky would take a suspension of endothelial cells—the cells that line the insides of blood vessels—and inject it into the vessel network. The cells would settle in and multiply to line the insides of the channels, effectively turning the channels into blood vessels. And then the cells would spread—they would begin to branch off the existing vessels and form new ones. In effect, Lewis and her team have created an environment that the cells consider home—it is far more natural to them than a petri dish or the inorganic scaffolds that had previously played host to cultured tissues.
“I like to say that we design the highway and then get out of the way and let the endothelial cells create their own driveways,” Lewis said. “It’s better to rely on the intelligence of the cells themselves in terms of how they like to sprout.”
Lewis’s approach is only one of many in the wider effort to create complex tissues. A team of researchers from Brigham and Women’s Hospital and Carnegie Mellon University are working toward building a magnetically controlled “micro-robot” that can arrange cells in pre-specified structures. Other groups, at Boston University, Rice University, and at M.I.T., are pursuing ways of 3-D-printing vascular channels using a sugar-based ink. “I think it’s wonderful,” Jordan Miller, of Rice, said of Lewis’s work. “She is a world leader.”
For her part, Lewis is passionate about the changes that 3-D printing could bring to the pharmaceutical industry. Billions of dollars each year are spent on drug development that fails. If bioprinted tissues were readily available, experimental drugs could be tested on them to see how the drugs are metabolized and what side effects result. “We want to provide a fail-fast model,” Lewis said, “so that drugs can be assessed in 3-D human tissue and their toxic properties identified before spending money and effort in animal and human testing.”
But Lewis admits that she does think about the prospect of printing whole, functioning organs. “The grand challenge is to make a whole kidney—that is our moon shot,” she said. The first step would be to make a nephron, the fundamental filtering unit of a kidney. She noted that her team has already demonstrated the ability to print any pattern of vascular channels that it wants; the blood-vessel patterns of a nephron are just one more option. And recently the team found a way to line channels with epithelial cells taken from human kidneys. “We see this as a ladder, one step following the next,” she said. “Creating just a single nephron would be a great achievement. But there are a million in each kidney.”
Before I left the lab, Kolesky showed me the incubator, a white box near the printer that was about the size of a dorm-room refrigerator. He opened the door. On a shelf, on a glass slide, was a finished block of printed tissue. A fine plastic tube, the size of a strand of spaghetti, entered it at one end, delivering glucose, amino acids, and other critical nutrients. Another spaghetti-like tube emerged from the other end, carrying off carbon dioxide, broken-down proteins, and other cellular waste. The tissue had been alive for the past two weeks, Kolesky said. There wasn’t much to see, but the tissue was thriving, and it looked to me like the start of something very big.
Kommentare
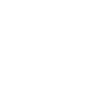